Stato delle applicazioni delle batterie agli ioni di litio
Crisi ambientale ed energetica
L'aggravarsi dei problemi ambientali e l'incombente crisi energetica hanno spinto il passaggio globale verso soluzioni energetiche sostenibili. Questa transizione sottolinea il ruolo critico dei nuovi veicoli energetici e delle tecnologie avanzate di stoccaggio dell'energia nel mitigare queste sfide pressanti. L'urgenza di questo cambiamento è ulteriormente amplificata dalla necessità di ridurre le emissioni di carbonio e la dipendenza dai combustibili fossili, che sono al centro delle attuali crisi ambientali ed energetiche.
I nuovi veicoli energetici, in particolare quelli alimentati da batterie agli ioni di litio, offrono una strada promettente per ridurre le emissioni di gas serra e combattere il cambiamento climatico. Questi veicoli non solo riducono la dipendenza dal petrolio, ma contribuiscono anche a rendere più pulita la qualità dell'aria, riducendo al minimo le emissioni allo scarico. Inoltre, lo sviluppo di tecnologie avanzate per l'immagazzinamento dell'energia, come le batterie agli ioni di litio ad alta capacità, è essenziale per bilanciare la natura intermittente delle fonti di energia rinnovabili come il solare e l'eolico.
L'integrazione di materiali anodici a base di silicio nelle batterie agli ioni di litio rappresenta un progresso significativo in questo campo. Il silicio, con la sua elevata capacità specifica teorica, offre miglioramenti sostanziali rispetto ai tradizionali anodi di grafite. Tuttavia, le sfide associate al silicio, come le grandi variazioni di volume durante la litizzazione/delitizzazione e la scarsa conduttività, richiedono soluzioni innovative. Il rivestimento in carbonio, ad esempio, è emerso come una strategia valida per aumentare la conduttività e la stabilità degli anodi di silicio, migliorando così le prestazioni complessive delle batterie agli ioni di litio.
In sintesi, la transizione energetica globale non è solo una risposta alle crisi ambientali ed energetiche, ma una strategia proattiva per costruire un futuro sostenibile. I progressi nei nuovi veicoli energetici e nelle tecnologie di stoccaggio dell'energia, in particolare quelli che coinvolgono i materiali a base di silicio, sono fondamentali in questo sforzo. Con la ricerca e lo sviluppo che continuano a spingere i confini del possibile, il potenziale per creare un ecosistema energetico più sostenibile e resiliente diventa sempre più raggiungibile.
Vantaggi delle batterie agli ioni di litio
Le batterie agli ioni di litio sono diventate la pietra miliare dei moderni sistemi di stoccaggio dell'energia, soprattutto grazie ai loro numerosi vantaggi. Uno dei vantaggi più significativi è il loro il basso impatto ambientale . A differenza delle tradizionali batterie al piombo, le batterie agli ioni di litio non contengono metalli pesanti tossici, riducendo il rischio di inquinamento durante la produzione, l'uso e lo smaltimento. Questa caratteristica ecologica le rende una scelta ideale per applicazioni che vanno dall'elettronica di consumo ai veicoli elettrici.
Inoltre, le batterie agli ioni di litio vantano una durata del ciclo eccezionalmente lunga . Possono essere sottoposte a centinaia, se non migliaia, di cicli di carica-scarica senza che le prestazioni si degradino in modo significativo. Questa longevità garantisce che i dispositivi alimentati da batterie agli ioni di litio rimangano funzionanti per lunghi periodi, riducendo la frequenza delle sostituzioni e i costi associati.
La alta densità di energia delle batterie agli ioni di litio è un altro fattore critico che ne determina la diffusione. Queste batterie possono immagazzinare una quantità sostanziale di energia in uno spazio compatto, il che le rende perfette per l'elettronica portatile e per i veicoli elettrici, dove spazio e peso sono fattori critici. L'elevata densità di energia si traduce in tempi di funzionamento più lunghi e in una maggiore autonomia di viaggio, migliorando l'esperienza e la convenienza dell'utente.
Oltre a questi vantaggi fondamentali, le batterie agli ioni di litio offrono capacità di ricarica rapida e bassi tassi di autoscarica . La ricarica rapida consente agli utenti di ripristinare rapidamente l'energia della batteria, mentre la bassa autoscarica garantisce che la batteria mantenga la carica anche in caso di lunghi periodi di inattività. Queste caratteristiche, unite al design leggero e all'elevata efficienza, rendono le batterie agli ioni di litio adatte a una miriade di applicazioni, dagli smartphone ai computer portatili, dai sistemi di accumulo di energia rinnovabile ai veicoli elettrici.
Limiti dell'anodo di grafite
La grafite, il materiale anodico più diffuso in commercio, presenta diverse limitazioni intrinseche che ne ostacolano le prestazioni nelle batterie agli ioni di litio. Uno degli svantaggi principali è la sua capacità specifica teorica relativamente bassa, che limita la quantità di ioni di litio che possono essere immagazzinati e, di conseguenza, la densità energetica complessiva della batteria. Questa limitazione è particolarmente significativa nel contesto della crescente domanda di soluzioni di accumulo di energia più elevate, come quelle necessarie per i veicoli elettrici e l'elettronica portatile.
Inoltre, la grafite presenta potenziali problemi di sicurezza a causa del suo basso potenziale di litio incorporato. Questa caratteristica può portare a problemi come la placcatura del litio, un fenomeno in cui il litio metallico si deposita in modo non uniforme sulla superficie dell'anodo durante la carica. La placcatura del litio non solo riduce l'efficienza della batteria, ma comporta anche un rischio significativo di cortocircuito, che può provocare il salto termico e, in casi estremi, il guasto della batteria o addirittura l'esplosione.
In sintesi, sebbene la grafite rimanga una pietra miliare nel settore delle batterie agli ioni di litio, i suoi limiti in termini di capacità e sicurezza rendono necessaria l'esplorazione e l'adozione di materiali alternativi, come gli anodi a base di silicio, che promettono di risolvere queste carenze e di aprire la strada alle tecnologie delle batterie di prossima generazione.
Materiali anodici a base di silicio
Vantaggi dell'anodo al silicio
Il silicio è un materiale anodico molto promettente per le batterie agli ioni di litio, soprattutto grazie alla sua eccezionale capacità specifica teorica. A differenza della grafite, che ha una capacità teorica di circa 372 mAh/g, il silicio vanta una capacità teorica significativamente più elevata, pari a circa 4200 mAh/g. Questo sostanziale aumento della capacità si traduce in una maggiore durata della batteria e in una maggiore densità energetica, rendendo gli anodi a base di silicio altamente desiderabili per le applicazioni che richiedono periodi operativi prolungati e design compatti.
Inoltre, il silicio presenta prestazioni di sicurezza superiori rispetto alla grafite. Gli anodi di grafite sono suscettibili di problemi di sicurezza, in particolare durante il processo di litizzazione, a causa del loro basso potenziale di litio incorporato. Al contrario, gli anodi di silicio dimostrano un comportamento elettrochimico più stabile e sicuro, riducendo il rischio di fuga termica e altri rischi di sicurezza associati alle batterie agli ioni di litio. Questo profilo di sicurezza migliorato è fondamentale per garantire l'affidabilità e la longevità dei sistemi di batterie, soprattutto in applicazioni ad alta richiesta come i veicoli elettrici e l'elettronica portatile.
In sintesi, la combinazione di un'elevata capacità specifica teorica e di migliori prestazioni di sicurezza fa del silicio un'alternativa superiore alla grafite nello sviluppo delle batterie agli ioni di litio di prossima generazione.
Le sfide dell'anodo di silicio
Gli anodi di silicio devono affrontare diverse sfide significative che ne ostacolano le prestazioni nelle batterie agli ioni di litio. Uno dei problemi principali è la sostanziale variazione di volume subita dal silicio durante il processo di delitizzazione. Questa espansione e contrazione volumetrica può portare alla degradazione meccanica del materiale, con conseguenti scarse prestazioni e rapido decadimento della capacità.
Inoltre, il silicio presenta una scarsa conducibilità elettrica, che aggrava ulteriormente i suoi limiti prestazionali. Per risolvere questi problemi, i ricercatori hanno esplorato diverse strategie, come la variazione della dimensionalità del silicio attraverso la nanostrutturazione. Questo approccio ha portato allo sviluppo di anodi di silicio gerarchici compositi, che sono diventati uno dei principali obiettivi per le applicazioni future.
All'inizio degli anni 2020, i progressi tecnologici hanno portato questi anodi compositi sull'orlo della commercializzazione, con la creazione di impianti di produzione di massa negli Stati Uniti. Nonostante questi promettenti sviluppi, la ricerca di un materiale anodico ottimale continua. Il litio metallico, ad esempio, offre una capacità specifica superiore a quella del silicio, ma presenta una serie di problemi, tra cui l'instabilità del litio metallico e il rischio di formazione di dendriti.
Lo strato intermedio di elettrolita solido (SEI) rimane una sfida critica per la progettazione, in particolare nel contesto degli anodi di litio metallico. Se stabilizzato con successo, il litio metallico potrebbe rivoluzionare la tecnologia delle batterie, offrendo la massima capacità di carica e mantenendo un profilo leggero.
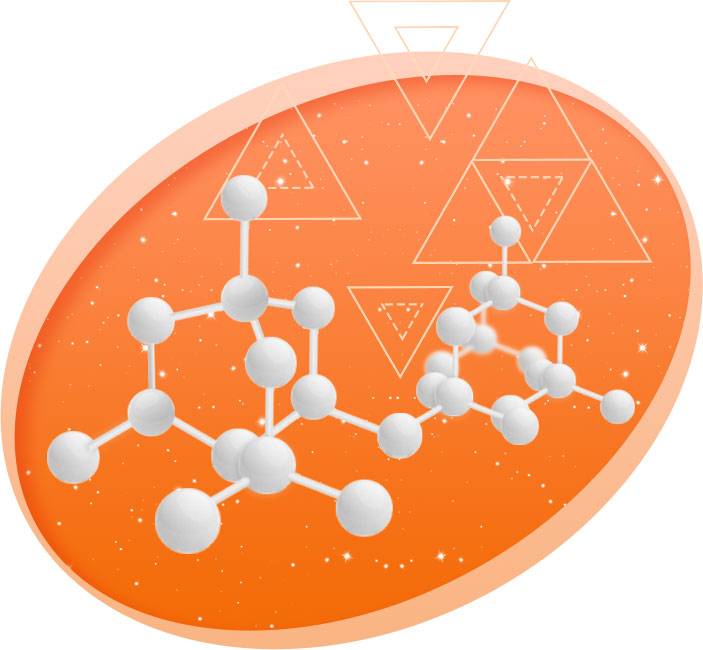
Strategie per mitigare le sfide
I ricercatori hanno implementato una serie di strategie innovative per affrontare le sfide intrinseche del silicio come materiale anodico nelle batterie agli ioni di litio. Queste strategie sono state progettate per aumentare la conduttività del silicio, mitigare la sua espansione di volume durante i cicli e migliorare le sue prestazioni elettrochimiche complessive.
Uno dei metodi principali prevede la nanostrutturazione che prevede la riduzione delle dimensioni delle particelle di silicio su scala nanometrica. Questo approccio sfrutta l'aumento dell'area superficiale e la riduzione delle lunghezze di diffusione su scala nanometrica, migliorando così la cinetica di inserimento ed estrazione degli ioni di litio. Inoltre, il silicio nanostrutturato è in grado di gestire meglio le grandi variazioni di volume associate alla litizzazione e alla delitizzazione, riducendo le sollecitazioni meccaniche e il rischio di frattura delle particelle.
Un'altra strategia critica è il rivestimento superficiale con elementi conduttivi . Il carbonio è il rivestimento conduttivo più utilizzato grazie alla sua eccellente conduttività elettronica e stabilità meccanica. I rivestimenti di carbonio possono essere applicati con varie tecniche, come la deposizione chimica da vapore (CVD), la macinazione a sfere ad alta energia e l'essiccazione a spruzzo. Questi rivestimenti fungono da strato protettivo, impedendo il contatto diretto tra il silicio e l'elettrolita, riducendo la formazione di strati indesiderati di interfase solida dell'elettrolita (SEI) e migliorando la stabilità ciclistica dell'anodo.
Inoltre, l'uso di strutture a matrice rigida per fornire un supporto meccanico e tamponare le variazioni di volume del silicio durante i cicli. Queste strutture a matrice, spesso composte da carbonio o altri materiali rigidi, possono incapsulare le particelle di silicio, offrendo una struttura stabile che mantiene l'integrità strutturale e previene la disintegrazione del materiale anodico.
Strategia | Descrizione | Benefici |
---|---|---|
Nanostrutturazione | Riduzione delle dimensioni delle particelle di silicio su scala nanometrica | Cinetica migliorata, migliore sistemazione della variazione di volume, riduzione della frattura |
Rivestimento superficiale | Applicazione di rivestimenti conduttivi (ad esempio, carbonio) al silicio | Maggiore conduttività, ridotta formazione di SEI, maggiore stabilità ai cicli |
Matrice rigida | Utilizzo di strutture rigide per incapsulare il silicio | Supporto meccanico, mantenimento dell'integrità strutturale, prevenzione della disintegrazione |
Queste strategie mirano complessivamente a sfruttare l'elevata capacità specifica teorica del silicio superandone i limiti, aprendo così la strada alla sua applicazione pratica nelle batterie agli ioni di litio di prossima generazione.
Metodi di preparazione dei compositi silicio-carbonio
Deposizione chimica da vapore (CVD)
La deposizione chimica da vapore (CVD) è una sofisticata tecnica di deposizione sotto vuoto utilizzata per generare materiali solidi di alta qualità e ad alte prestazioni. Questo processo è ampiamente utilizzato nell'industria dei semiconduttori per la deposizione di film sottili, fondamentali per migliorare le prestazioni dei dispositivi. In una tipica configurazione CVD, il substrato, spesso un wafer di silicio, viene esposto a uno o più precursori volatili. Questi precursori subiscono reazioni chimiche o decomposizione sulla superficie del substrato, dando origine al rivestimento desiderato. Contemporaneamente, si generano sottoprodotti volatili che vengono successivamente rimossi attraverso il flusso di gas all'interno della camera di reazione.
La CVD è particolarmente vantaggiosa per rivestire il silicio con il carbonio, un processo che migliora significativamente le caratteristiche del ciclo dei materiali a base di silicio nelle batterie agli ioni di litio. Il rivestimento di carbonio non solo migliora la conducibilità elettrica del silicio, ma ne tampona anche le forti variazioni di volume durante i cicli di litizzazione e delitizzazione. Nonostante questi vantaggi, la CVD è caratterizzata da un'elevata complessità e da costi elevati, che possono essere attribuiti all'intricata configurazione e alla necessità di un controllo preciso dei vari parametri di processo.
La versatilità della CVD è ulteriormente dimostrata dalla sua capacità di depositare un'ampia gamma di materiali in forme diverse, tra cui strutture monocristalline, policristalline, amorfe ed epitassiali. Questi materiali vanno dai composti di silicio come il biossido di silicio, il carburo, il nitruro e l'ossinitruro, ai materiali avanzati di carbonio come le fibre di carbonio, le nanofibre, i nanotubi, il diamante e il grafene. Inoltre, la CVD è in grado di depositare metalli come il tungsteno e il nitruro di titanio, nonché dielettrici ad alta densità, rendendola uno strumento versatile nei processi di microfabbricazione.
Lo sviluppo della CVD risale al 1960, quando John M. Blocher, Jr. coniò il termine per differenziarla dalla Physical Vapor Deposition (PVD). Nel corso degli anni sono stati sviluppati vari formati di CVD, ognuno dei quali si differenzia per il modo in cui vengono avviate le reazioni chimiche. Ad esempio, la CVD termica a parete calda opera in modalità batch, mentre la CVD assistita da plasma sfrutta il plasma per migliorare il processo di deposizione. Queste variazioni sottolineano l'adattabilità della CVD, che la rende una pietra miliare nella produzione di materiali avanzati per diverse applicazioni, comprese le tecnologie di stoccaggio dell'energia come le batterie agli ioni di litio.

Macinazione a sfere ad alta energia
La macinazione a sfere ad alta energia è un metodo robusto ed efficiente per la preparazione di compositi silicio-carbonio, in particolare per l'uso nelle batterie agli ioni di litio. Questa tecnica sfrutta l'elevata energia cinetica delle sfere o delle perle di macinazione per frantumare e mescolare i materiali, facilitando una rapida riduzione delle dimensioni e la formazione di leghe meccaniche. Il processo è particolarmente efficace per i materiali duri e fragili come metalli, leghe, ceramiche e minerali, il che lo rende una scelta ideale per i materiali anodici a base di silicio.
Vantaggi principali
- Ciclo di produzione breve: Una delle caratteristiche principali della macinazione a sfere ad alta energia è la sua capacità di lavorazione rapida. L'azione di macinazione intensa e ad alta velocità consente di produrre rapidamente particelle fini, riducendo significativamente il tempo necessario per la preparazione del materiale.
- Efficiente dal punto di vista dei costi: Rispetto ad altri metodi come la deposizione chimica da vapore (CVD), la macinazione a sfere ad alta energia è più economica. Le attrezzature necessarie sono meno complesse e i costi operativi sono inferiori, il che la rende un'opzione finanziariamente interessante per le applicazioni industriali.
Limitazioni e sfide
- Contenuto di silicio: Nonostante la sua efficienza, la macinazione a sfere ad alta energia ha dei limiti per quanto riguarda il contenuto di silicio. Il processo può portare a danni strutturali e alla degradazione delle particelle di silicio, a scapito delle prestazioni complessive del materiale anodico.
- Danno strutturale: L'impatto ad alta energia può causare uno stress meccanico significativo, portando alla formazione di difetti e crepe nelle particelle di silicio. Questi danni strutturali possono compromettere l'integrità e le prestazioni elettrochimiche del prodotto finale.
Applicazione nelle batterie agli ioni di litio
La macinazione a sfere ad alta energia è particolarmente utile nel contesto delle batterie agli ioni di litio, dove la necessità di materiali finemente macinati e uniformemente miscelati è fondamentale. Il metodo può produrre compositi di silicio-carbonio con un'elevata area superficiale e siti reattivi, fondamentali per migliorare le prestazioni delle batterie. Tuttavia, le sfide inerenti al contenuto di silicio e ai danni strutturali richiedono un'attenta ottimizzazione ed eventualmente l'integrazione di ulteriori tecniche di modifica della superficie per mitigare questi problemi.
In sintesi, mentre la macinazione a sfere ad alta energia offre una via promettente per la preparazione rapida ed economica di compositi silicio-carbonio, è essenziale affrontare i suoi limiti per sfruttare appieno il suo potenziale nella produzione di materiali anodici avanzati per le batterie agli ioni di litio.
Essiccazione a spruzzo
L'essiccazione a spruzzo è un metodo fondamentale per la preparazione di compositi silicio-carbonio (Si/C), in particolare per la loro applicazione come materiali anodici nelle batterie agli ioni di litio. Questa tecnica offre diversi vantaggi distinti che contribuiscono alle prestazioni complessive e alla stabilità dell'anodo.
Uno dei vantaggi principali dell'essiccazione a spruzzo è la capacità di produrre compositi con elevata ritenzione di capacità . Questo è fondamentale per garantire che l'anodo mantenga la sua capacità di trasporto della carica per più cicli, un fattore critico per la longevità delle batterie agli ioni di litio. Il metodo consente di ottenere questo risultato controllando attentamente la formazione della struttura composita, che aiuta a ridurre al minimo l'affievolimento della capacità.
Inoltre, l'essiccazione a spruzzo facilita la creazione di una struttura di pori adeguata all'interno dei compositi Si/C. Questa struttura di pori è essenziale per il materiale anodico, poiché migliora l'infiltrazione dell'elettrolita e il trasporto degli ioni all'interno del materiale. Un migliore trasporto degli ioni porta a migliori prestazioni elettrochimiche, tra cui tempi di ricarica più rapidi e un più efficiente stoccaggio dell'energia.
Il processo prevede la nebulizzazione di una soluzione precursore in goccioline sottili, che vengono poi essiccate per formare particelle solide. Queste particelle vengono successivamente trattate termicamente per formare il composito Si/C finale. L'ambiente controllato durante l'essiccazione a spruzzo consente di regolare con precisione le dimensioni e la morfologia delle particelle, che sono fondamentali per ottimizzare le prestazioni del materiale.
In sintesi, l'essiccazione a spruzzo è un metodo versatile ed efficace per produrre compositi di Si/C che presentano un'elevata ritenzione di capacità e una struttura ideale dei pori, rendendoli molto adatti all'uso come materiali anodici nelle batterie agli ioni di litio.
Metodo idrotermale
Il metodo idrotermale è una tecnica sofisticata utilizzata per sintetizzare i compositi silicio-carbonio, che prevede processi di evaporazione idrotermale ad alta temperatura. Questo metodo sfrutta le proprietà uniche delle condizioni idrotermali per creare compositi che presentano un'eccezionale stabilità ai cicli, in particolare quando vengono incorporati additivi di carburo di vanadio (VC).
Durante il processo idrotermale, l'ambiente ad alta temperatura facilita la formazione di legami chimici stabili tra silicio e carbonio, dando vita a un materiale composito adatto all'uso nelle batterie agli ioni di litio. L'incorporazione di additivi VC migliora ulteriormente la stabilità dei cicli di questi compositi, fornendo una struttura più robusta in grado di resistere ai ripetuti cicli di carica e scarica senza subire degradazioni significative.
Questo metodo è particolarmente vantaggioso per la sua capacità di produrre compositi con un alto grado di uniformità e integrità strutturale. Le condizioni di alta temperatura assicurano che i compositi siano privi di difetti, il che è fondamentale per mantenere le prestazioni a lungo termine del materiale anodico nelle batterie agli ioni di litio. Inoltre, l'uso dell'evaporazione idrotermale consente un controllo preciso della microstruttura del composito, essenziale per ottimizzare le sue proprietà elettrochimiche.
In sintesi, il metodo idrotermale offre una via promettente per la preparazione di compositi silicio-carbonio con un'eccellente stabilità ai cicli, rendendola una tecnica preziosa nella ricerca in corso per lo sviluppo di materiali anodici avanzati per le batterie agli ioni di litio.
Metodo Sol-Gel
Il metodo sol-gel è una tecnica versatile e ampiamente utilizzata per la fabbricazione di film sottili e altri materiali, in particolare nel contesto degli anodi a base di silicio per le batterie agli ioni di litio. Questo metodo prevede la formazione di una sospensione colloidale, nota come "sol", che passa a una fase solida, simile a un gel. Questa transizione è facilitata da una serie di reazioni di idrolisi e polimerizzazione, che danno luogo a una macromolecola solida e stabile immersa in un solvente.
Uno dei principali vantaggi del metodo sol-gel è la sua capacità di operare a temperature sperimentali relativamente basse, il che lo rende una scelta pratica per i ricercatori. Inoltre, il processo è semplice e facile da gestire e richiede un'attrezzatura specializzata minima. Tuttavia, questi vantaggi hanno un costo. Il metodo sol-gel è noto per i suoi alti costi di sintesi e i lunghi tempi di lavorazione, che possono rappresentare uno svantaggio significativo, soprattutto nelle applicazioni industriali dove l'efficienza e l'economicità sono fondamentali.
Vantaggi | Svantaggi |
---|---|
Bassa temperatura sperimentale | Alto costo di sintesi |
Facilità di funzionamento | Tempi di lavorazione lunghi |
Nonostante queste sfide, il metodo sol-gel rimane uno strumento prezioso per la preparazione di compositi silicio-carbonio, offrendo un equilibrio tra controllo e flessibilità difficilmente raggiungibile con altri metodi.
Metodo a microonde
La sintesi a microonde è emersa come una tecnica promettente per la produzione di materiali in silicio rivestiti di grafene, che presentano eccezionali proprietà elettrochimiche. Questo metodo sfrutta la capacità unica delle microonde di riscaldare rapidamente i materiali, portando alla formazione di strati di grafene di alta qualità su substrati di silicio. I materiali di silicio rivestiti di grafene che ne risultano dimostrano un'elevata capacità specifica reversibile, fondamentale per migliorare le capacità di accumulo di energia delle batterie agli ioni di litio.
Uno dei vantaggi principali del metodo a microonde è la capacità di produrre materiali con una stabilità ciclistica superiore. Ciò si ottiene grazie al riscaldamento uniforme e rapido fornito dalle microonde, che favorisce la formazione di un rivestimento di grafene stabile e conduttivo. Questo rivestimento non solo migliora la conduttività complessiva dell'anodo a base di silicio, ma attenua anche i problemi di espansione di volume comunemente associati al silicio durante i processi di litizzazione e delitizzazione.
Vantaggi | Descrizione |
---|---|
Elevata capacità reversibile | Il rivestimento in grafene aumenta la capacità specifica del silicio, migliorando l'accumulo di energia. |
Buona stabilità del ciclo | Il riscaldamento uniforme garantisce la stabilità dello strato di grafene, migliorando le prestazioni del ciclo. |
Attenuazione dell'espansione del volume | Il rivestimento conduttivo aiuta a tamponare le variazioni di volume del silicio, riducendo il decadimento della capacità. |
Il metodo a microonde è particolarmente interessante per i tempi di sintesi relativamente brevi e per la capacità di produrre materiali di qualità costante. Queste caratteristiche lo rendono un'alternativa competitiva ad altre tecniche di rivestimento del carbonio, come la deposizione chimica da vapore (CVD) e la macinazione a sfere ad alta energia, che spesso comportano processi più complessi e lunghi.
In sintesi, il metodo di sintesi a microonde offre un approccio semplificato alla creazione di materiali di silicio rivestiti di grafene, adatti alle applicazioni avanzate delle batterie agli ioni di litio. Combinando l'elevata capacità del silicio con le proprietà conduttive e protettive del grafene, questo metodo apre la strada allo sviluppo di materiali anodici di prossima generazione con prestazioni e stabilità migliorate.
Sintesi in situ
La sintesi in situ è un metodo sofisticato che catalizza la crescita di strati di carbonio direttamente sulle nanoparticelle di silicio. Questa tecnica è particolarmente vantaggiosa per aumentare la forza del legame interfacciale tra silicio e carbonio, fondamentale per migliorare le prestazioni elettrochimiche complessive degli anodi a base di silicio nelle batterie agli ioni di litio.
Il processo prevede la deposizione controllata di atomi di carbonio sulla superficie del silicio, creando un'interfaccia robusta e conduttiva. Questa interfaccia non solo attenua le variazioni di volume che il silicio subisce durante i cicli di litizzazione e delitizzazione, ma migliora anche in modo significativo la conduttività elettrica del materiale. Di conseguenza, il composito silicio-carbonio mostra una stabilità superiore nei cicli e una maggiore ritenzione di capacità rispetto agli anodi di silicio tradizionali.
Inoltre, il metodo di sintesi in situ consente di regolare con precisione lo spessore e la struttura dello strato di carbonio, che può essere ottimizzato per migliorare ulteriormente le prestazioni dell'anodo. Questo livello di personalizzazione è particolarmente vantaggioso per affrontare le sfide specifiche associate agli anodi di silicio, come la loro scarsa conduttività e il rapido decadimento della capacità.
In sintesi, la sintesi in situ offre un percorso promettente per lo sviluppo di anodi compositi silicio-carbonio ad alte prestazioni, fornendo una soluzione solida ai limiti dei materiali convenzionali a base di silicio nelle batterie agli ioni di litio.
CONTATTATECI PER UNA CONSULENZA GRATUITA
I prodotti e i servizi di KINTEK LAB SOLUTION sono stati riconosciuti da clienti di tutto il mondo. Il nostro personale sarà lieto di assistervi per qualsiasi richiesta. Contattateci per una consulenza gratuita e parlate con uno specialista del prodotto per trovare la soluzione più adatta alle vostre esigenze applicative!